The golf boom in the United States after World War II stimulated many practical changes in putting green construction. One of the adjustments is the increased use of sand as an amendment to the native push-up greens or using pure sand as root-zone material.
As a result of the research conducted in the 1950s, sand-based green specifications were generated by the USGA Green Section. These specifications have been revised several times since they were first published. The uniqueness of USGA greens is the inclusion of a gravel layer below the sand profile, which creates a hanging or perched water table. With an optimum size of sand particles, the root zone can provide sufficient air space and remain less vulnerable to compaction. The artificially created water table helps hold water, and the amount might be increased further by adding organic materials or inorganic soil amendments to the sand.
To meet USGA specifications, the material has to satisfy certain standards. Presently, USGA recommends total porosity be 35 to 55 percent, noncapillary porosity, 15 to 30 percent, and capillary porosity, 15 to 25 percent. The current USGA-recommended saturated water conductivity is at least 6 inches per hour.
It’s important the material is tested before, during and after the construction of the putting greens for contracting, quality control and inspection purposes. Testing physical properties starts with particle size analysis, which dictates other properties. The confidence interval for particle size analysis is about 10 percent to around 35 percent. For water conductivity, it’s about 20 percent using the USGA-specified procedures. The confidence interval is used to compare differences between loads as they’re mixed, and for quality control purposes. For instance, if a standard drains at 10 inches per hour, then each subsequent load should drain between 8 and 12 inches per hour, based on the 20 percent confidence interval for saturated water conductivity (Ksat).
The inconsistency of those test results for root-zone materials within the labs has caused inconvenience in bidding and contracting processes during construction. At times, architects, contractors and superintendents didn’t know how to use the results, so they applied much more stringent criteria than the Ksat tests were able to meet. Saturated water conductivity has been a magic phrase among golf course superintendents when they talk about greens. Saturated water flow occurs only for a short period during a rain or irrigation event.
Conductivity is only a fraction of the water movement characterizing root-zone materials. More information is needed about the unsaturated flow of water to understand the root-zone materials better. A superintendent also might want to know how saturated water conductivity changes throughout time and how it’s affected by cultural practices.
The primary objective of this study was to develop a methodology that allows easy measurement and monitoring of water conductivity of root zones without the need for destructive sampling. Some academic exercises also were involved to investigate factors that influence the accuracy and consistency of saturated water conductivity tests, such as the soil packing process, dissolved air in testing water, wetting direction and organic matter.
Measuring methods
Two sand sources were included in the study (Table 1, at left). Sand I conformed to USGA specifications, and Sand II was higher in the fine fraction. The soil materials were packed into brass rings at a moisture condition of 8 percent.
A calcium sulfate-thymol solution was prepared following the procedure by Klute and Dirksen. Thymol was used to inhibit the growth of microorganisms in the solution. Before the solution was used, dissolved air was removed with a vacuum boiling apparatus.
Before testing capillary porosity, water retention curve and water conductivity, the soil samples were saturated at normal atmospheric pressure or under a vacuumed condition with three test solutions: tap water, deionized water and a calcium sulfate-thymol solution. Because water conductivity increases exponentially with degree of saturation, a small variation at the saturating point can cause dramatic differences in saturated conductivity. The purpose of these procedures was to test if improvements made in the degree of saturation can help improve the consistency of saturated water conductivity measurements. Tap water can disperse soil aggregates and cause underestimation of water conductivity, so calcium sulfate was used to increase the concentration of testing water.
Using deionized water or a calcium sulfate-thymol solution didn’t seem to improve the consistency in the measurement of water holding capacity and conductivity. One reason might be the low clay content and lack of soil structure in the testing materials. Saturating the soil material under reduced pressure generally provided higher estimation of water conductivity (Table 2, page 87) without much improvement in consistency. This led us to believe other random errors induced during the sample preparation and testing process might be underlying reasons for the poor repeatability. Because sand particle size distribution is fundamentally responsible for pore size distribution and water conductivity, it’s important to have accurate estimation of the particle size analysis. This is especially true when organic materials are incorporated in the root zone because a thorough mixing is difficult to achieve.
Although there were large variations in saturated water conductivity, it might not be as problematic as commonly considered from an agronomic point of view. Saturated flow rarely happens under actual putting green conditions, and if it happens, it’s in a different way than that of the laboratory test where saturation starts from the bottom of the sample.
Furthermore, saturated water conductivity decreases quickly as the green ages because of fine particle migration, fine organic material accumulation and layering. Essentially, good drainage is maintained through diligent cultural practices, provided correct materials were used during construction and for topdressing.
Including a water release curve in specified tests can be useful to bridge the gap between perception and reality. Water release curves provide more balanced information about hydraulic properties of the root-zone materials in addition to water holding capacity and saturated water conductivity. Instead of debating which pressure heads should be used to determine air porosity, water release curves allow the end user to interpret water holding capacity and air porosity based on the root-zone depth.
A separate study was conducted to test the hypothesis of whether putting greens that vary in depth can provide water regime control. As shown in Figure 1, when the water release curve is rotated 90 degrees, total air and water volumes, as indicated in the figures, are determined by root-zone depth – 0 being the bottom of the root zone. Contrary to the traditional air porosity report, which reflects only the average across the depth of soil core being used in the test, using the whole water release curve can provide information of air and water in the whole profile.
Water content and air capacity can be predicted from the water release curve using the van Genuchten equation. Graphically, the water capacity is the area to the right of the curve enclosed by the curve, axis, and soil depth line, while the air capacity is the area to the left of the curve enclosed by the curve, top line of water content and line of soil depth. In this case, the total water and air capacity is 20 percent and 10 percent, instead of 10 percent and 20 percent, respectively, for the 40-cm-deep root zone. The water content and air capacity would be 25 percent and 5 percent, instead of 17 percent and 13 percent, respectively, for the 20-cm-deep root zone.
Measuring water infiltration
A tension infiltrometer equipped with a differential transducer as described by Casey and Derby was used to measure water infiltration in the lab and in the field. The transducer calibration was conducted on a suction table from saturation to 350 mm, in 10-mm increments. A linear regression equation (R2=0.99) was achieved between the voltage reading and the tension setting. Water tension at the bottom of the disk was monitored from the transducer by closing the water inlet briefly and then checked against the flow rate of water.
Soil samples also were packed in brass rings 10 cm in diameter and 10 cm tall to a bulk density of 1.55 g/cm3. Water infiltration was tested on the repacked soil cores and in the field using a time domain reflectometer (TDR)-tension infiltrometer. Materials were tested on 300, 250, 120, 60, 30, 20, 10, and 0 mm tension settings, 10 minutes for the first four settings and 5 minutes for the last four settings. The transducer was logged every second for the first 1 minute and every 2 seconds afterward. Water conductivity for 3-D infiltration in the field was calculated following a nonlinear regression method. Water conductivity of one-dimensional infiltration was calculated by the method described by Klute and Dirksen.
The tensions at the bottom of the infiltration disk were close to the set tensions except minor differences for Sand I. The discrepancy was attributed to the high flow rate at near saturation. The problem can be corrected through increasing the diameter of the connecting tube from the water reservoir to the infiltration disk and reducing friction loss of the pressure head from the valves and fittings.
With inclusion of water measuring probes, such as TDR, the water content can be measured during the same process of measuring water conductivity. The whole process can be automated to measure and estimate the major soil hydraulic properties at the same time with the same set up, reducing human error and operation time.
Tensiometers were built with the same principle as described by Ankeny, et al. Two dimensions of the infiltration disk were manufactured, 10 cm and 20 cm in diameter. The three-rod probes are 86 cm long, 0.25 cm in diameter and spaced 1.5 cm apart. The performance of TDR probe was evaluated with a TDR-100 with water and air, respectively. The wave form provided enough resolution for precise measurement of water depth, which was calculated from L minus x, where L is the TDR rod length, and x is the distance of water surface to the top of the water supplying tower.
Sand materials were prepared in a PVC tube 10 cm in diameter and 7.8 cm in length with a double layer of cheese cloth attached at the bottom with a rubber band. At the side of the PVC tube, three access holes were drilled to insert a three-rod TDR probe 5 cm long. Both TDR probes in the soil and the TDR probe in the infiltrometer were multiplexed via a SDMX50 multiplexer to a data logger.
The performance of TDR automated water level measurement was compared with differential pressure transducer automated and visual observation. Water level measurement automated with TDR is as good as, or better than, differential pressure transducer automated measurements.
Water content and infiltration were measured at 10-cm water tension. Soil sorptivity for the laboratory materials was estimated using the differentiated linearization method developed by Vandervaere et al. Saturated water conductivities were calculated according to this method as well. Water release curve could be established from the tension and water content in the soil cores. Alternatively, air porosity was derived from the water content and bulk density measurement at the 30-cm tension set on the infiltrometer. Saturated water conductivity and air porosity data are shown in Table 3.
Water retention and conductivity
Using the tension infiltrometer equipped with TDR for water-level monitoring, we were able to monitor water level without the need for calibration for each measurement, which is required for transducers. Water content in the sample immediately below the infiltrometer also was measured with a TDR probe at the same time infiltration was measured. Thus, the soil water retention and water conductivity can be measured simultaneously, whereas in the traditional procedures, water retention and water conductivity are measured in two separate steps.
The following points highlight the differences between the TDR-equipped infiltrometer approach and the traditional approach specified in the ASTM methods:
- The TDR-infiltrometer method uses core soil samples 10 cm in diameter – twice as big as in traditional methods. The TDR-infiltrometer method has less error introduced by marginal flow effects.
- Because water retention and water conductivity are measured simultaneously, compaction of soil samples after water retention measurements and before water conductivity measurement as in the traditional procedures is avoided, which greatly reduces variations associated with compacting.
- Measurements of the TDR-infiltrometer method are conducted in the unsaturated range of soil samples, which reduces the inconsistency of saturation that contributes to major vatiation in the water conductivity measurement.
- Wetting direction in the TDR-infiltrometer method is the same for laboratory and field samples. It also can be used to monitor water conductivity in situ in the field and allows direct agronomic interpretation and comparison of laboratory test results.
- Devices used in traditional methods usually are fabricated by individual laboratories, while the TDR-infiltrometer method uses a more accurate, specially manufactured instrument. The initial cost can be quickly offset by savings in labor and time.
Confidence intervals of the water holding and water conductivity test results can be reduced among and within laboratories. Soil hydraulic properties from the laboratory test can be compared with the field performance because of the consistent methodology. The TDR-infiltrometer method also can be used to collect soil water movement information to be used for subsurface irrigation control and estimation of chemical movement within soil profiles. GCI
Deying Li, Ph.D., is an associate professor in the department of plant sciences at North Dakota State University in Fargo.
Credit: USGA Turfgrass and Environmental Research Online, Volume 7, Number 7, April 1, 2008.
Literature Cited
1. Ankeny, M.D., M. Ahmed, T.C. Kaspar, and R. Horton. 1991. Simple field method for determining unsaturated hydraulic conductivity. Soil Sci. Soc. Am. J. 55:467-470.
2. American Society for Testing and Materials. 1998. Standard test methods for saturated hydraulic conductivity, water retention, porosity, particle density, and bulk density of putting green and sports turf root zones. Annual Book of ASTM Standards. Vol 15.07. ASTM designation:F1815-97. Philadelphia, PA. (TGIF Record 96236)
3.Casey, F.X.M,. and N.D.Derby. 2002. Improved design for an automated tension infiltrometer. Soil Sci. Soc. Am. J. 66:64-67.
4. Davis, W.B., J.L.Paul and D.Bowman. 1990. The sand putting green: construction and management. Publication No.21448. University of California Division of Agriculture and Natural Resources. (TGIF Record 14801)
5. Klute, A., and C.Dirksen. 1986. Hydraulic conductivity and diffusivity: Laboratory methods. Pages 687-734. In A.Klute (ed.) Methods of Soil Analysis. Part 1. 2nd Ed. Agron. Mongr. 9. ASA and SSSA, Madison, WI. (TGIF Record 57217)
6. Li, D., D. D. Minner, N. E. Christians, and S. Logsdon. 2005. Evaluating the impact of variable root-zone depth on the hydraulic properties of sand-based turf systems. Int. Turfgrass Soc. Res. J. 10: . (TGIF Record 106349)
7. Logston, S.D., and D.B. Jaynes. 1993. Methodology for determining hydraulic conductivity with tension infiltrometers. Soil Sci. Soc. Am. J. 57:1426-1431.
8. USGA Green Section Staff. 1993. USGA recommendations for a method of putting green construction. USGA Green Section Record 31(2):1-3. (TGIF Record 26681)
9. Vandervaere, J.P., C. Peugeot, M. Vauclin, and R. A. Jaramillo. 1997. Estimating hydraulic conductivity of crusted soils using disc infiltrometers and minitensiometers. J. Hydrology 188-189:203-23.
10. van Genuchten, M. Th., F. J. Leij, and S. R. Yates. 1991. The RETC codes for quantifying the hydaulic functions of unsaturated soils, Version 1.0. EPA Report 600/2-91/065, U.S. Salinity Laboratory, USDA, ARS, Riverside, California.
11. van Genuchten, M. Th. 1980. A closed-form equation for predicting the hydraulic conductivity of unsaturated soils. Soil Sci. Soc. Am. J. 44:892-898.
Get curated news on YOUR industry.
Enter your email to receive our newsletters.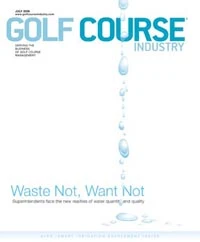
Explore the July 2008 Issue
Check out more from this issue and find your next story to read.
Latest from Golf Course Industry
- Advanced Turf Solutions’ Scott Lund expands role
- South Carolina’s Tidewater Golf Club completes renovation project
- SePRO to host webinar on plant growth regulators
- Turfco introduces riding applicator
- From the publisher’s pen: The golf guilt trip
- Bob Farren lands Carolinas GCSA highest honor
- Architect Brian Curley breaks ground on new First Tee venue
- Turfco unveils new fairway topdresser and material handler